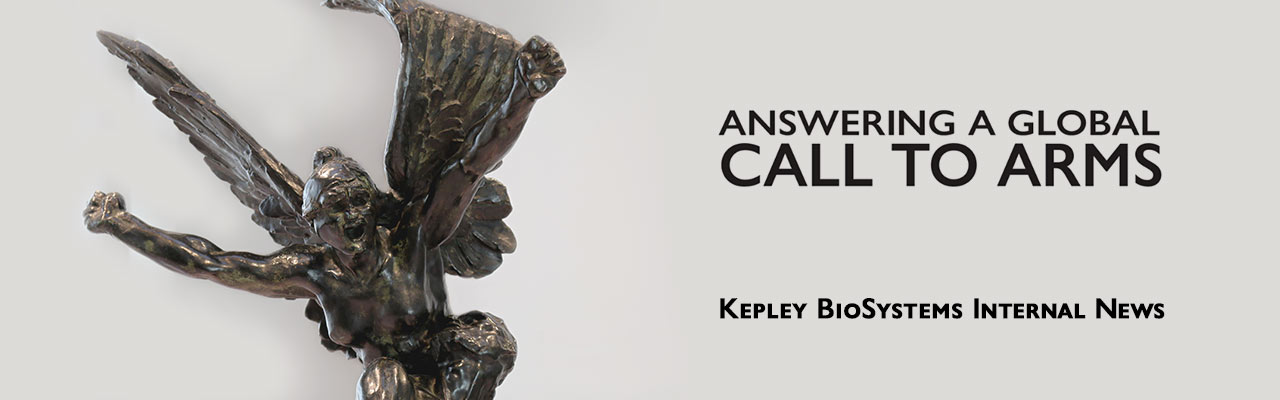
Answering a Global Call to Arms
Antimicrobial resistance, or AMR, is regarded as one of the most dire threats to mankind. As such, related diseases could take ten million lives annually by 2050 while consuming some $100 trillion of global GDP (1,2). Overall, 300 million people are expected to die prematurely as a result of AMR over the next 35 years(1). Appropriate, timely treatment of bacterial infections is nonetheless a formidable task, as future efficacy comes into question due to suboptimal or unnecessary drug administration. In turn, every instance of delayed detection and management increases the risk of infection (septicemia) advancing to sepsis. In turn, a “call to arms” has established that by 2020, antibiotic use must be predicated on data and testing technologies(2).
Nearly 50 years ago, remarkable reactivity to the majority of the most threatening microorganisms at parts per trillion was discovered in the cells of the horseshoe crab, an ancient and familiar arthropod. Early attempts to apply this discovery to detection of septicemia were abandoned due to apparent interfering substances in human specimens, yet it was ensconced as the gold standard for the most demanding sterility testing in medical device and injectables quality control. Over time, habitat erosion, biomedical capture and bleeding, and dissection of horseshoe crabs as whelk and eel bait have gradually threatened wild populations of this once ubitquitous species and therefore, a vital resource for ensuring drug and device safety, worldwide.
Given the global threat posed by gram-negative bacteria and the horseshoe crab cellular response to them, Kepley BioSystems set out to answer the “call to arms” by challenging the status quo. With National Science Foundation grant funding, the North Carolina biotech sought to establish a sustainable supply of this crucial raw material with specialized aquaculture systems, nutritional management, surgical catheter implantation and low-impact, routine hemolymph collection methods. All milestones were achieved: the horseshoe crabs have appeared to thrive and have yielded highly reactive cells.
With feasibility funding from North Carolina Sea Grant and in affiliation with the Joint School of Nanoscience and Nanoengineering (JSNN) laboratories, the team then set its sights on elimination of patient specimen interferences and endotoxin test standardization. Feasibility was achieved using cells derived from the aquaculture cohort. The biotech is now seeking investors to address the AMR crisis through sustainable resource scale-up (and species conservation) and to commercialize a simple assay that could obviate protracted culturing as well as incremental risks from unnecessary interventions.
A Massive Threat from Microorganisms
Bacteria have been humanity’s most pervasive predators, with particular virulence of antibiotic resistant pathogens emerging throughout the world. To date, the World Health Organization (WHO) has listed and ranked 12 virulent species as: Critical, High or Medium in importance (4). Nine are gram-negative, yet the list omits a frequent cause of public health advisories, E. Coli, which is also gram-negative, accounting for 10 of 13 of the most daunting threats. Primary care physicians initiated half of the 260 million outpatient antibiotic prescriptions dispensed in the US in 2011 (5). Whereby, about 80% of the population receives an average of one per year, of which at least 30% are not needed(6). Some diagnostics, such as urine dipsticks for proteinuria, can contribute to this phenomenon. One recent study found some 83% of patients (n = 2,733) with asymptomatic bacteriuria received a full course of antibiotics (7).
Public health professionals have emphasized stewardship for years, insisting on optimal use to improve patient outcomes while preventing multi-drug resistance (8). However, the lack of rapid, definitive results often leads to inappropriate administration, as illustrated by the latter example.
Sepsis by the Numbers
Annually, some 30 million people worldwide are diagnosed with sepsis; it is the third leading cause of death overall, outpacing prostate and breast cancer and HIV/AIDs combined, with a 25-30% mortality rate(9-12). About one-third of such patients enter the health care system through emergency rooms, representing an enormous hospital liability(13). It is also the leading cost of hospitalization ($24 billion annually) and the primary cause of US re-admissions, with 19% rehospitalization within 30 days(9,14,15). The incidence has been steadily rising 1.5% per year, and related costs have increased nearly 20% since 2011(14,16).
Early Diagnosis and Treatment Saves Lives
Early sepsis diagnosis and treatment improves patient survival(17) and reduces the cost of care; whereby, mortality rates decrease by 7.6% per hour with appropriate administration of antibiotics(18). However, accepted laboratory methods have not advanced substantially in 140 years since the advent of culture techniques. Rapid, comparably sensitive analytical options have remained an elusive means to: transform the way antimicrobials are prescribed; reduce unnecessary use; slow the trajectory of AMR; and extend drug viability.
Discoveries and Challenges Associated with Alternatives to Blood Culture
In the 1970s, the discovery of the instantaneous response to gram-negative pathogens using horseshoe crab (Limulus Polyphemus) cells by Jack Levin and Frederik Bang(19) promised immediate endotoxin results (the “Holy Grail” of sepsis testing) in human blood using Limulus amebocyte lysate (LAL). Still, the LAL assay was regrettably and quickly found problematic, with reports of considerable variability due to interfering substances causing false positives and negatives(20). In 1970 and 1971, Levin also published two manuscripts discussing such observations(21,22). These anomalies were initially attributed to enzymatic degradation of lipopolysaccharides (LPS)(23-26); while some surmised that LPS was binding to serum and plasma proteins(27,28); and others later suggested alpha-2-macroglobulin(29), LPS antibodies(30) and high-density lipoproteins as contributing factors(31,32).
Three leading institutions had studied and renounced LAL by 1974: Tilton Lab at the University of Connecticut(33); McCabe Lab at Boston University Hospital(34-36); and the Feldman and Pearson Lab at St. Jude(37). These collaborators asserted that, “the Limulus amebocyte lysate test is not clinically useful for detecting gram-negative infections or predicting their outcome. Lack of standardized procedures for lysate preparation and endotoxin extraction from plasma could explain the current disagreement of published test results”(37). By 1975, Ronald Elin and his colleagues at the National Institutes of Health sounded the “death knell” for the LAL assay as a diagnostic test in The New England Journal of Medicine, reporting on a “Lack of Clinical Usefulness of the Limulus Amebocyte Test in Diagnosis of Endotoxemia”(38).
Limulus Amebocyte Lysate Assay Adapted for Drug and Device Sterility Testing
Thereafter, James Cooper adapted the LAL assay for pharmaceutical and device quality control, which garnered FDA approval(39). It became the biomedical industry test of choice for gram-negative endotoxins due to simplicity, specificity, and sensitivity(40). LAL is also reactive to fungal glucans (1→3-β-D-Glucan; BDG), which have become increasingly important both clinically and environmentally given recent Candida auris outbreaks(41). BDG makes up a significant portion of the cell wall of most fungi, including clinically relevant species such as Aspergillus, Candida, Fusarium, and Pneumocystis. With respect to clinically significant applications, it should be noted that one of the quality control LAL testing market leaders developed a protocol for human specimens in the 1990s; however, procedural complexities, and perhaps the cost or risk of limited LAL resources, nonetheless appear to have relegated it to a research method without adoption in human medicine(42).
A Detour from Human Medicine
This detour from human medicine perpetuated a dearth of objective diagnostic and prognostic tools(43). While not yet modified for clinical use, LAL is nonetheless the most sensitive, rapid method for bacterial endotoxin detection(44) with specificity for 70-80% of pathogens typically attributed to sepsis(8). Solving the human blood interference issues could enable physicians to initiate more informed, potentially lifesaving therapy using a clinical LAL assay.
Reliable LAL screening could nonetheless detect a gram-negative infection without known etiology, much as the reference method of culturing has done for more than a century(45,46). Still, an overarching “bloodborne infection” determination would define treatment options from a battlefield, to a rural clinic to a university hospital. The nuances of pathogen identity and antibiotic sensitivity do not alter the clinical imperative to treat immediately while awaiting additional information in an era of dangerous pathogens – while avoiding the perils of unnecessary drug use.
As mentioned at the outset, applications for LAL assays in human medicine have also been deemed ill-advised due to resource constraints stemming from declining horseshoe crab populations needed to produce the reagents. Early examination of those fed enriched diets in captivity has also suggested possible malnourishment in the wild due to diminishing habitat feedstocks, accelerated by rising sea temperatures and encroachment from urban development(47).
The Quest to Develop LAL Assays for Human Medicine
For the North Carolina biotech team, seeking breakthroughs to address species sustainability and clinical specimen integrity issues ultimately represented scientific and humanitarian imperatives: First, to secure and expand the supply of raw material by developing sustainable horseshoe crab aquaculture, harvesting and nutritional management strategies. Second, to achieve LAL assay reliability by overcoming interferences in human blood (i.e. reproducibility, sensitivity and specificity).
Establishing a Framework for Sustainable LAL
The team first hypothesized that: Optimized aquaculture, nutritional management and bleeding strategies for horseshoe crabs would lead to sustainable production of LAL. Initially, the literature was dissuasive regarding captive husbandry for biomedical assays. Most previous attempts failed and were ascribed to circadian, lunar, tidal and other rhythms presumed to be interrupted by removal from an open habitat(48). Those notions proved fallacious.
Following the award of a National Science Foundation grant, calm, apparently thriving horseshoe crabs were maintained in a controlled aquaculture system with enriched feedstock and consistent oxygen saturation in safe surroundings. They were also healthy before, during and after surgical implantation of a patent-pending catheter that facilitated low-impact cell harvesting. These efforts yielded rapid animal recovery, routine bleeding feasibility (~12-24 times annually) and highly reactive LAL (>160% vs. commercial components analyzed during the study). All normal behaviors have been observed and continued without apparent stress or decline in wellbeing since October 2018. Similarly, an array of biomarkers from aquacultured horseshoe crabs reared on in-house functional feed formulations also suggested that nutrition was an essential factor as compared to results when first collected, as well as the kits derived from wild capture(47).
This work allowed for optimization of the aquaculture parameters, feedstock formulation and catheter techniques to establish the framework for a sustainable supply of LAL, largely addressing its potential for clinical use.
A Reliable Sepsis/Septicemia Screening Method
The second hypothesis proposed that: Removal of interfering substances would allow for detection of LPS in patient specimens. Preliminary research suggested that LAL could detect pathogens and unbound endotoxins in heretofore “impossible substrates” (i.e. human blood). Specifically, the aquaculture-derived LAL yielded reproducibility from 6 CFU/mL to 625,000 CFU/mL in spiked arrays with intact gram-negative bacteria and < 5.0 EU/mL with free LPS(47). These ranges and performance characteristics align with established practice standards, from asymptomatic septicemia to sepsis. As such, LAL clinical screening could outperform culture and PCR methods in speed, labor and cost.
Disruptive Innovation with Global Impact
In summary, horseshoe crab aquaculture has been shown to be practicable with respect to viability, quality, raw material, and environmental and economic considerations. In addition, feasibility of a method for LAL detection of gram-negative bacteria in clinical settings has been shown to overcome previously erratic results with human blood specimens. These developments directly address the global imperatives(1-3). However, the North Carolina biotech, Kepley BioSystems, lacks sufficient resources to commercialize this technology and is seeking guidance to help bring these innovations forward.
Given such means, sustainable aquaculture and year-round, low-impact harvesting could be rapidly scaled up with a single, permanent cohort representing a fraction of the vast, random-capture burden now placed on wild populations year after year. In turn, a rapid, gram-negative bacteria screening product for human sepsis could be available upon completion of a collaborative clinical study. Ultimately, a simple blood-based LAL assay could be performed across the spectrum of clinical settings while saving potentially lethal hours awaiting culture results or raising the specter of AMR from overzealous treatment.
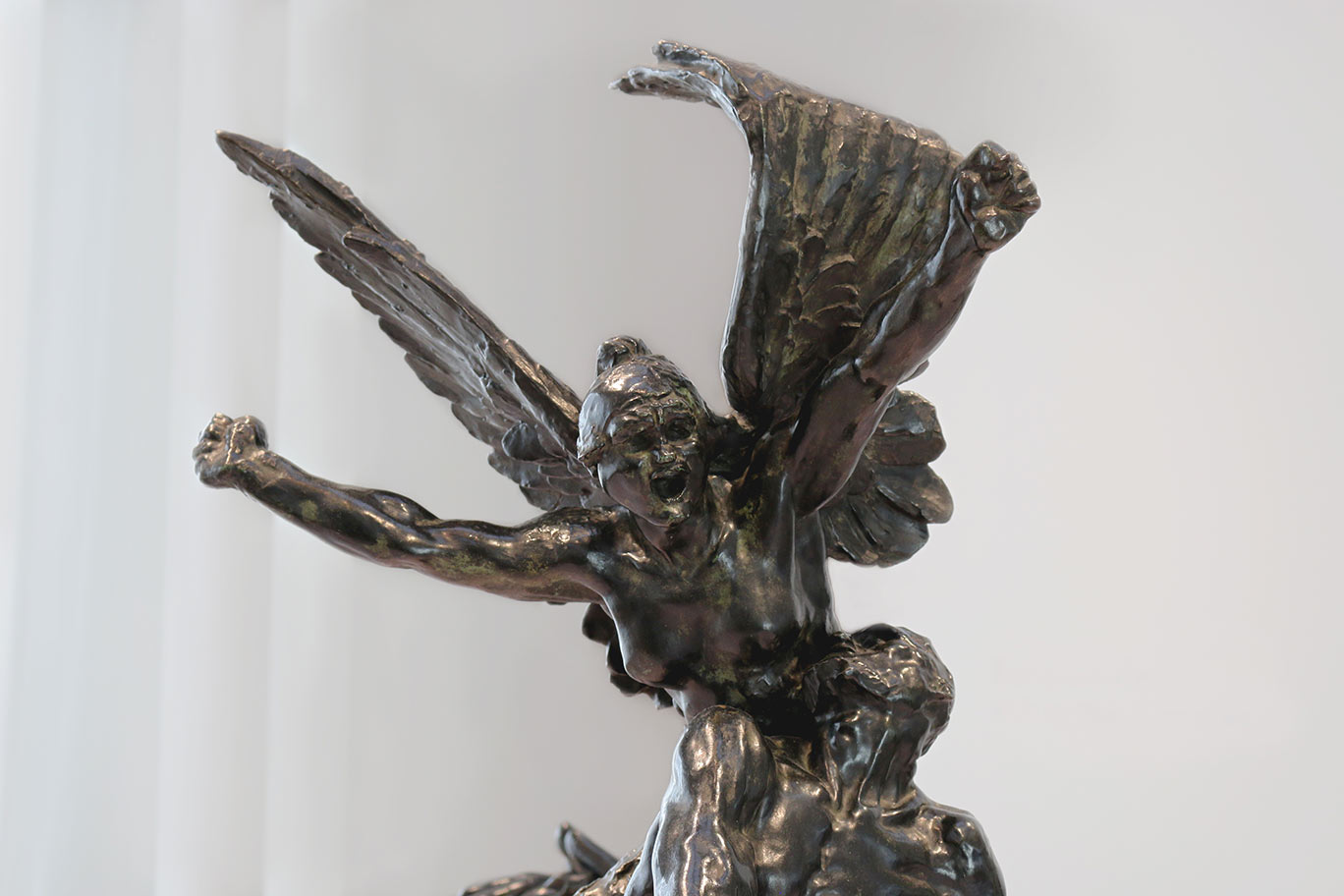
Auguste Rodin. L’Appel Aux Armes (The Call to Arms).
Modeled 1879, cast at a later date.
Permanent Collection of North Carolina Museum of Art
References
- O’Neill J. (2015). The review on antimicrobial resistance 2015 - Securing new drugs for future generations: The pipeline of antibiotics. The Review on Antimicrobial Resistance. Report commissioned by the UK Prime Minister. Published – May 2015.
- O’Neill J. (2017). Tackling drug-resistant infections globally: Final report and recommendations. London: Review on Antimicrobial Resistance; Published – May 2016.
- O’Neill J. (2014). Antimicrobial resistance: Tackling a crisis for the health and wealth of a nation. London: Review on Antimicrobial Resistance; Published – December 2014.
- World Health Organization. (2017). WHO publishes list of bacteria for which new antibiotics are urgently needed. Chicago.
- Hicks LA, Bartoces MG, Roberts RM, et al. (2015). US outpatient antibiotic prescribing variation according to geography, patient population, and provider specialty in 2011. Clinical Infectious Disease; 60:1308–16.
- Fleming-Dutra KE, Hersh AL, Shapiro DJ, et al. (2016). Prevalence of inappropriate antibiotic prescriptions among US ambulatory care visits, 2010-2011. The Journal of the American Medical Association (JAMA); 315(17): 1864-73.
- Petty LA., Vaughn VM, Flanders SA, et al. (2019). "Risk factors and outcomes associated with treatment of asymptomatic bacteriuria in hospitalized patients." The Journal of the American Medical Association (JAMA) Intern Med (Opinion: Published Online – August 26, 2019).
- MacVane SH. (2017). Antimicrobial resistance in the intensive care unit: A focus on gram-negative bacterial infections. Journal of Intensive Care Medicine; 32(1), 25-37.
- Sepsis Alliance. (2018). Fact sheet 2018. Sepsis.org. Available online: https://www.sepsis.org/faq/attachment/r1-sepsis-fact-sheet-2018/. Accessed on October 13, 2019.
- Center for Disease Control and Prevention (CDC). (2017). Making healthcare safer: Think sepsis. Available online: https://www.cdc.gov/vitalsigns/pdf/2016-08-vitalsigns.pdf. Accessed on October 13, 2019.
- Kumar G, Kumar N, Taneja A, et al. (2011). Nationwide trends of severe sepsis in the twenty first century (2000-2007). Chest;16:1223–1231.
- Hall MJ, Williams SJ, DeFrances CJ, Golosinsky A. (2011). Inpatient care for septicemia or sepsis: a challenge for patients and hospitals. Available online: http://www.cdc.gov/nchs/data/databriefs/db62.pdf. Accessed on October 13, 2019.
- Perman SM., Goyal M, Gaieski D F. (2012). Initial emergency department diagnosis and management of adult patients with severe sepsis and septic shock. Scandinavian Journal of Trauma, Resuscitation and Emergency Medicine;20,41.
- Fingar K. (2015). Trends in hospital readmissions for four high-volume conditions, 2009-2013. Available online: https://www.hcup-us.ahrq.gov/reports/statbriefs/sb196-Readmissions-Trends-High-Volume-Conditions.jsp. Accessed on October 13, 2019.
- Torio C, Moore B. (2016). National inpatient hospital costs: The most expensive conditions by payer. Available online: http://www.hcup-us.ahrq.gov/reports/statbriefs/sb204-Most-Expensive-Hospital-Conditions.pdf. Accessed on October 13, 2019.
- Angus DC, Linde-Zwirble, WT, Lidicker J, et al. (2001). Epidemiology of severe sepsis in the United States: analysis of incidence, outcome, and associated costs of care. Critical Care Medicine; 29(4), 1303-10.
- Berg D, Gerlach H. (2018). Recent advances in understanding and managing sepsis. F1000 Research; 7. Available online: https://www.ncbi.nlm.nih.gov/pmc/articles/PMC6173111/. Accessed on October 13,2019.
- Kumar A, Roberts D, Wood KE et al. (2006). Duration of hypotension before initiation of effective antimicrobial therapy is the critical determinant of survival in human septic shock. Critical Care Medicine; 34(6), 1589–96.
- Levin, J. (2019). Discovery and early development of the limulus test. In endotoxin detection and control in pharma, limulus, and mammalian systems. Springer, Cham; 3-16.
- Hurley, JC. (1994). Concordance of endotoxemia with gram-negative bacteremia in patients with gram-negative sepsis: A meta-analysis. Journal of Clinical Microbiology; 32(9), 2120-2127.
- Levin J, Tomasulo PA, Oser RS. (1970). Detection of endotoxin in blood and demonstration of an inhibitor. Journal of Laboratory and Clinical Medicine; 75, 903-911.
- Levin J, Poore TE, Zauber NP, Ose RS. (1971). Detection of endotoxin in the blood of patients with sepsis due to gram-negative bacteria. New England Journal of Medicine; 283, 1313-1316.
- Rowley D, Ali N, Jenkin CR. (1958). A reaction between fresh serum and lipopolysaccharides of gram-negative Rowley D, Ali N, Jenkin CR. (1958). A reaction between fresh serum and lipopolysaccharides of gram-negative bacteria. British Journal of Experimental Pathology; 39, 90–98.
- Keene WR, Landy M, Shear MJ. (1961).: Inactivation of endotoxin by a humoral component VII: enzymatic degradation of endotoxin by blood plasma. Journal of Clinical Investigation; 40, 302–310.
- Skarnes RC. (1966). The inactivation of endotoxin after interaction with certain proteins of normal serum. Annals of the New York Academy of Sciences; 133, 644–662.
- Skarnes RC. (1968). In vivo interaction of endotoxin with a plasma lipoprotein having esterase activity. Journal of Bacteriology; 95, 2031–2034.
- Oroszlam S, McFarland VW, Mora PT, Shear MJ. (1966). Reversible inactivation of an endotoxin by plasma proteins. Annals of the New York Academy of Sciences; 133, 622–628.
- Rudbach JA, Anacker RL, Haskins WF, et al. (1966). Physical aspects of reversible inactivation of endotoxin. Annals of the New York Academy of Sciences; 133, 629–643.
- Yoshioka M, Konno S. (1984). Characteristics of endotoxin altering fractions derived from normal serum III. Isolation and properties of horse serumα 2-macroglobulin. Journal of Laboratory and Clinical Medicine;104, 321–330.
- Wardle N. (1979). Bacteraemic and endotoxic shock. British Journal of Hospital Medicine; 21, 223–231.
- Freudenberg MA, Bøg-Hansen TC, Back U, Galanos L. (1980). Interaction of lipopolysaccharides with plasma high-density lipoprotein in rats. Infection and Immunity; 28, 373–380.
- Ulevitch RJ, Johnston AR, Weinstein DB. (1981). New function for high density lipoproteins: isolation and characterization of a bacterial-lipopolysaccharide-high density lipoprotein complex formed in rabbit plasma. Journal of Clinical Investigation; 67, 827–837.
- Martinez LA, Quintiliani R, Tilton RC. (1973). Clinical experience on the detection of endotoxemia with the limulus test. Journal of Infectious Disease;1 27(1), 102-105.
- McCabe WR, Kreger BE, Johns M. (1972). Type-specific and cross-reactive antibodies in gram-negative bacteremia. New England Journal of Medicine; 287(6), 261-267.
- Stumacher RJ, Kovnat MJ, McCabe WR. (1973). Limitations of the usefulness of the Limulus assay for endotoxin. New England Journal of Medicine; 288(24), 1261-1264.
- Zinner SH, McCabe WR. (1976). Effects of IgM and IgG antibody in patients with bacteremia due to gram-negative bacilli. Journal of Infectious Disease; 133(1), 37-45.
- Feldman S, Pearson TA. (1974). The Limulus test and gram-negative bacillary sepsis. American Journal of Diseases of Children; 128(2), 172-174.
- Elin RJ, Robinson RA, Levine AS, Wolff SM. (1975). Lack of clinical usefulness of the limulus test in the diagnosis of endotoxemia. New England Journal of Medicine; 293(11), 521-524.
- Hochstein, HD. Role of the FDA in regulating the Limulus amoebocyte lysate test. Clinical applications of the Limulus amoebocyte lysate test (1990).
- Cooper JF. Bacterial endotoxins test. In: Prince R, ed. Microbiology in pharmaceutical manufacturing. Godalming, United Kingdom: Davis Horwood International; 537-67.
- Gnauck, A., Lentle, R. G., & Kruger, M. C. (2016). Chasing a ghost?–Issues with the determination of circulating levels of endotoxin in human blood. Critical reviews in clinical laboratory sciences, 53(3), 197-215.
- Roslansky PF, Novitsky TJ. (1991). Sensitivity of limulus amebocyte lysate (LAL) to LAL-reactive glucans. Journal of Clinical Microbiology; 29(11), 2477-2483.
- Vincent JL. (1997). Dear SIRS, I'm sorry to say that I don't like you. Critical Care Medicine; 25(2), 372-374.
- Hurley JC, Nowak P, Öhrmalm L, et al. (2015). Endotoxemia as a diagnostic tool for patients with suspected bacteremia caused by gram-negative organisms: A meta-analysis of four decades of studies. Journal of Clinical Microbiology; 53(4), 1183-1191.
- Libman, E. (1906). On some experiences with blood-cultures in the study of bacterial infections. Bulletin of the Johns Hopkins Hospital; 6, 17:215-28.
- Libman E. (1906). The etiology of subacute infective endocarditis. American Journal of the Medical Sciences; 140, 516-26.
- Data on file. Kepley BioSystems Inc. (2019).
- Smith DR, Mandt MT, MacDonald PD (2009). Proximate causes of sexual size dimorphism in horseshoe crabs (limulus polyphemus) of the Delaware Bay. Journal of Shellfish Research; 28(2), 405-418.